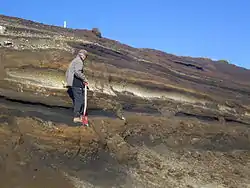
A tephra layer which bears its own unique chemistry and character may be used as a temporal marker horizon in archaeological and geological sites.
Tephra
'Tephra' is fragmental material produced by a volcanic eruption regardless of composition, fragment size, or emplacement mechanism.[1]
The distribution of tephra following an eruption usually involves the largest boulders falling to the ground quickest and therefore closest to the vent, while smaller fragments travel further. Ash can often travel for thousands of miles, even circumglobal, as it can stay in the stratosphere for days to weeks following an eruption. When large amounts of tephra accumulate in the atmosphere from massive volcanic eruptions (or from a multitude of smaller eruptions occurring simultaneously), they can reflect light and heat from the sun back through the atmosphere, in some cases causing the temperature to drop, resulting in a climate change: "volcanic winter". Tephra mixed in with precipitation can also be acidic and cause acid rain and snowfall.
Tephra fragments are classified by size:
- Volcanic ash (Ash) - particles smaller than 2 mm (0.08 inches) in diameter,
- Lapilli or volcanic cinders - between 2 and 64 mm (0.08 and 2.5 inches) in diameter, and
- Volcanic bombs or volcanic blocks - larger than 64 mm (2.5 inches) in diameter.
Stratum
In geology and related fields, a 'stratum' (plural: 'strata') is a layer of rock or soil with internally consistent characteristics that distinguish it from other layers. Each layer is generally one of a number of parallel layers that lie one upon another, laid down by natural forces. They may extend over hundreds of thousands of square kilometers of the Earth's surface. Strata are typically seen as bands of different colored or differently structured material exposed in cliffs, road cuts, quarries, and river banks. Individual bands may vary in thickness from a few millimeters to a kilometer or more. Each band represents a specific mode of deposition: river silt, beach sand, coal swamp, sand dune, lava bed, etc.
Isochron dating
'Isochron dating' is a common technique of radiometric dating and is applied to date certain events, such as crystallization, metamorphism, shock events, and differentiation of precursor melts, in the history of rocks. Isochron dating can be further separated into mineral isochron dating and whole rock isochron dating; both techniques are applied frequently to date terrestrial and also extraterrestrial rocks (meteorites). The advantage of isochron dating as compared to simple radiometric dating techniques is that no assumptions are needed about the initial amount of the daughter nuclide in the radioactive decay sequence. Indeed the initial amount of the daughter product can be determined using isochron dating. This technique can be applied if the daughter element has at least one stable isotope other than the daughter isotope into which the parent nuclide decays.
Magnetostratigraphy
Magnetostratigraphy is a chronostratigraphic technique used to date sedimentary and volcanic sequences. The method works by collecting oriented samples at measured intervals throughout the section. The samples are analyzed to determine their detrital remnant magnetism (DRM), that is, the polarity of Earth's magnetic field at the time a stratum was deposited. For sedimentary rocks, this is possible because when very fine-grained magnetic minerals (< 17 micrometres) fall through the water column, they orient themselves with Earth's magnetic field. Upon burial, that orientation is preserved. The minerals behave like tiny compasses. For volcanic rocks, magnetic minerals that form as the melt cools orient with the ambient magnetic field.
Oriented paleomagnetic core samples are collected in the field; mudstones, siltstones, and very fine-grained sandstones are the preferred lithologies because the magnetic grains are finer and more likely to orient with the ambient field during deposition. If the ancient magnetic field was oriented similar to today's field (North Magnetic Pole near the North Rotational Pole) the strata retain a normal polarity. If the data indicate that the North Magnetic Pole was near the South Rotational Pole, the strata exhibit reversed polarity.
Results of the individual samples are analysed by removing the natural remanent magnetization (NRM) to reveal the DRM. Following statistical analysis the results are used to generate a local magnetostratigraphic column that can then be compared against the Global Magnetic Polarity Time Scale.
This technique is used to date sequences that generally lack fossils or interbedded igneous rocks. The continuous nature of the sampling means that it is also a powerful technique for the estimation of sediment accumulation rates.
Soil horizon
A 'soil horizon' is a specific layer in the land area that is parallel to the soil surface and possesses physical characteristics which differ from the layers above and beneath. Horizon formation (horizonation) is a function of a range of geological, chemical, and biological processes and occurs over long time periods. Soils vary in the degree to which horizons are expressed. Relatively new deposits of soil parent material, such as alluvium, sand dunes, or volcanic ash, may have no horizon formation, or only the distinct layers of deposition. As age increases, horizons generally are more easily observed. The exception occurs in some older soils, with few horizons expressed in deeply weathered soils, such as the oxisols in tropical areas with high annual precipitation.
Identification and description of the horizons present at a given site is the first step in classifying a soil at higher levels, through the use of systems such as the USDA soil taxonomy or the Australian Soil Classification. The World Reference Base for Soil Resources lists 40 diagnostic horizons.[2]
The term 'horizon' describes each of the distinctive layers that occur in a soil. Each soil type has at least one, usually three or four horizons and these are described by soil scientists when seeking to classify soils (Soil-Net). Horizons are defined in most cases by obvious physical features, colour and texture being chief among them. These may be described both in absolute terms (particle size distribution for texture, for instance) and in terms relative to the surrounding material, i.e., ‘coarser’ or ‘sandier’ than the horizons above and below.
Most soils conform to a similar general pattern of horizons, often represented as an ‘ideal’ soil in diagrams. Each main horizon is denoted by a capital letter, which may then be followed by several alphanumerical modifiers highlighting particular outstanding features of the horizon. While the general O-A-B-C-R sequence seems fairly universal, some variation exists between the classification systems in different parts of the world. In addition, the exact definition of each main horizon may differ slightly – for instance, the US system uses the thickness of a horizon as a distinguishing feature, while the Australian system does not. It should be emphasised that no one system is more correct – as artificial constructs, their utility lies in their ability to accurately describe local conditions in a consistent manner.
Marker horizon
'Marker horizons' or 'chronohorizons' or 'marker beds' are stratigraphic units of the same age and of such distinctive composition and appearance, that, despite their presence in separate geographic locations, there is no doubt about their being of equivalent age (isochronous) and of common origin. Such clear markers facilitate the correlation of strata.
The ejecta from volcanoes and bolide impacts create useful markers, as different volcanic eruptions and impacts produce beds with distinctive compositions. Marker horizons of tephra are used as a dating tool in archaeology, since the dates of eruptions are generally well-established.
One particular bolide impact 65 million years ago, Chicxulub, is controversially held to have led to a major extinction event and produced an iridium anomaly that occurs in a thin, global layer of clay marking the Cretaceous–Tertiary boundary. [3][4] Iridium layers are associated with bolide impacts and are not unique, but when occurring in conjunction with the extinction of specialised tropical planktic foraminifera and the appearance of the first Danian species, signal a reliable marker horizon for the K–T boundary.[5]
Fossil faunal and floral assemblages, both marine and terrestrial, make for distinctive marker horizons. Some marker units are distinctive by virtue of their magnetic qualities. The Water Tower Slates, forming part of the Hospital Hill Series in the Witwatersrand Basin, include a fine-grained ferruginous quartzite which is particularly magnetic. From the same series a ripple-marked quartzite and a speckled bed are used as marker horizons.
Marker horizons may be created by sedimentologists and limnologists in order to measure deposition and erosion rates in a marsh or pond environment. The materials used for such an artificial horizon are chosen for their visibility and stability and may be brick dust, grog, sand, kaolin, glitter or feldspar clay.[6]
Archaeological horizon
An 'archaeological horizon' is a widely disseminated period of common art and artifacts at an archaeological site or, more usually, over a larger geographic area, and is a distinctive level in that site's or area's archaeological sequence.[7]
An example of an archaeological horizon is the Dark Earth horizon in England, which separates Roman artifacts from later native artifacts and which indicates the abandonment of urban areas in Roman Britain during the 2nd century.
The term is used to denote a series of stratigraphic relationships that form an archaeological phase or are part of the process of determining the archaeological phases of a site. An archaeological horizon can be understood as a break in contexts formed in the Harris matrix, which denotes a change in epoch on a given site by delineation in time of finds found within contexts.
The term 'Archaeological horizon' is sometimes, and somewhat incorrectly, used in place of the term layer or strata.
Tephrochronology
The use of tephra layers, which bear their own unique chemistry and character, as temporal marker horizons in archaeological and geological sites is known as 'tephrochronology'.
Bentonites
'Bentonite' is an absorbent aluminium phyllosilicate, in general, impure clay consisting mostly of montmorillonite. Bentonite usually forms from the weathering of volcanic ash, most often in the presence of water. In stratigraphy and tephrochronology, completely devitrified (weathered volcanic glass) ash-fall beds are commonly referred to as K-bentonites when the dominant clay species is illite]]. Other common clay species, and sometimes dominant, are montmorillonite and kaolinite. Kaolinite-dominated clays are commonly referred to as tonsteins and are typically associated with coal.
Synchronized dating
When attempting to date and cross-correlate phenomena in ice cores, for example, it is most helpful to find volcanic horizons which contain particles with compositions unique to specific volcanoes and dated, or dateable, eruptions.
The year 2000 is being used for synchronized dating of tephra layers and reporting 14C dates around the world. A year such as 1980 becomes 20 years before 2000 or 20 b2k.[8]
The "western United States [has] individual tephra layers [that have been dated] from the following tuffs and ash beds: (A) Rockland (0.575 Ma), Lava Creek B (0.639 Ma), and Bishop (0.774 Ma); (B) Huckleberry Ridge (2.09 Ma); (C) Upper Horse Hill (3.10 Ma), Nomlaki (3.34 Ma), and Putah (3.4 Ma); (D) Kilgore (4.45 Ma) and Lawlor (4.83 Ma); (E) Pinole (5.2–5.4 Ma), Wolverine Creek (5.59 Ma), Silver Peak Range (6.1 Ma), Roblar (6.26 Ma), Blacktail Creek (6.70 Ma), Zammoroni Quarry (7.26 Ma), Faust (7.49), Roblar (9.36 Ma), Hazen (9.81 Ma), CPT XV (10.5 Ma), Ibex Hollow (11.93 Ma), and CPT V (12.07 Ma) (4–6, 30–32)."[9]
Mount St. Helens
Mount St. Helens' is an active stratovolcano in Skamania County, Washington, in the Pacific Northwest region of the United States. The volcano is located in the Cascade Range and is part of the Cascade Volcanic Arc, a segment of the Pacific Ring of Fire that includes over 160 active volcanoes. This volcano is well known for its ash explosions and pyroclastic flows.
Mount St. Helens is most famous for its catastrophic eruption on May 18, 1980, at 8:32 am PDT[10] (20 b2k) which is the deadliest and most economically destructive volcanic event in the history of the United States. It is an example of a plinian eruption. A massive debris avalanche triggered by an earthquake measuring 5.0 on the Richter scale, caused the eruption, reducing the elevation of the mountain's summit from 9,677 ft (2,950 m) to 8,365 ft (2,550 m) and replacing it with a 1 mile (1.6 km) wide horseshoe-shaped crater. A sudden surge of magma from the Earth's mantle caused the earthquake.[11]
Mount St. Helens is geologically young compared with the other major Cascade volcanoes. It formed only within the past 40,000 years, and the pre-1980 summit cone began rising about 2,200 years ago.[12] The volcano is considered the most active in the Cascades within the Holocene epoch (the last 10,000 or so years).[13]
The plinian deposit from the May 18, 20 b2k, eruption shows a break-in-slope (thickness vs. distance) at about 27 km from source.[14] This break is too far from source to be explained by the transition from column margin to umbrella cloud sedimentation.[14] The most distal segment is composed of low Reynolds number particles.[14]
Ice cores from the Upper Fremont Glacier (UFG) in Wyoming, USA, taken in 1981 and 1980, 600 km from the volcano and directly upwind of the UFG, have a mercury containing tephra layer from the 20 b2k Mount St. Helens eruption.[15] The volcanic ash blanketed the region.[15]
Tephra set W
Eruption of the dacitic set W began the Kalama period late in the 15th century, probably in 520 b2k. The initial event produced the large-volume, pumiceous layer Wn, the second largest Holocene tephra from Mount St. Helens. Layer Wn is overlain by several smaller pumiceous tephras, including the moderate-volume layer We. Both layers Wn and We have been traced for hundreds of kilometers downwind.[16]
Using contiguous sampling, magnetic susceptibility measurements, wet sieving, light microscopy, and electron microprobe analysis of glass in pumice fragments, the 518-519 b2k Mount St. Helens We tephra layer is identified in sediments from Dog Lake in southeastern British Columbia (some 650 km away), suggesting that the plume drifted further north than previously thought.[17]
The GISP2 based calendrical age of Mount St. Helens Wn tephra dates the eruption that produced the Wn tephra layer at 520-521 b2k.[18]
Tephra set Y
The set Y eruptions started shortly after 4,000 b2k and continued at least to about 3,300 b2k.[16] The tephra set consists chiefly of two voluminous coarse pumice layers: Yn, the largest volume of Holocene tephra known from Mount St. Helens, and Ye.[16] Both have been found several hundred km downwind.[16]
A notable frost, tree ring event occurred in 4035 b2k among subalpine bristlecone pine observed at localities from California to Colorado, over a distance of some 1,300 km, that is attributed to Mount St. Helens.[19] This is the most severe frost event in the entire tree-ring record, as it occurs in all trees sampled and caused severe anatomical damage.[20] But, there does not appear to be a corresponding acidity peak in Greenland.[19] The frost-ring date does coincide approximately with a large radiocarbon-dated eruption of Mount St. Helens.[21]
Tephra set J
Eruptions between about 10,500 and 12,000 b2k deposited dacitic pumice layers near the volcano, recognized out to hundreds of km east.[16]
Following tephra set J is a dormant period lasting between about 4,000 b2k to 10,500 b2k.[16]
The mid-Holocene is a period of inactivity at Mount St. Helens.[17]
Tephra set S
Eruptions from about 13,000 b2k during the early Swift Creek stage deposited a few large-volume dacitic pumice layers near the volcano recognized up to hundreds of km east.[16]
Another mostly dormant interval occurred between 14,000 and 19,000 b2k.[16]
Tephra set M
About 20,500 b2k there are a set of tephra layers none of which is more than a few mm thick near the volcano.[16] Nevertheless, one ash bed has been recognized in Nevada.
Tephra set C
During the Ape Canyon stage (36,000 - 50,000 b2k) there is a set of tephra layers that contains at least two large-volume dacitic pumice layers. One erupted near the end of the Ape Canyon stage, records one of the largest volume tephra eruptions known to Mount St. Helens, and has been recognized as far away as Nevada.[16]
There is a dormant interval between the Ape Canyon and Cougar stage eruptions of about 15,000 yrs (21,000 - 36,000 b2k).[16]
Mount Tambora
'Mount Tambora' (or Tamboro) is an active stratovolcano, or composite volcano, on the island of Sumbawa, Indonesia. After a large magma chamber inside the mountain filled over the course of several decades, volcanic activity reached a historic climax in the super-colossal eruption of April 1815 (185 b2k).[22]
This eruption is rated 7 on the Volcanic Explosivity Index (VEI), the only such eruption since the Lake Taupo Hatepe eruption in about 180 AD.[23] With an estimated ejecta volume of 160 km3, Tambora's 1815 outburst is the largest volcanic eruption in recorded history. The giant eruption acidified the atmosphere for more than two years. This tephra layer, or acidity signal, in Greenland is relatively weak, because the eruptions happened south of the equator, but has been found in Dye 3 and other Greenland ice cores.[24]
See also
- Camp Century
- Greenland ice cores
- Illite
- Large igneous provinces
- Large volume volcanic eruptions in the Basin and Range Province
- List of largest volcanic eruptions
- List of large volcanic eruptions
- w:List of tephra layers
- NEEM Camp
- North Greenland Ice Core Project
- Renland
- Supervolcano
- Timetable of major worldwide volcanic eruptions
- Volcanic Explosivity Index
- Water
References
- ↑ This is the broad definition of tephra (Greek tephra, "ash") proposed by the Icelandic volcanologist Sigurður Þórarinsson (Sigurdur Thorarinsson) in 1954, in connection with the eruption of Hekla (Thorarinsson, "The eruption of Hekla, 1947-48II, 3, The tephra-fall from Hekla, March 29th, 1947", Visindafelag Islendinga (1954:1-3).
- ↑ World Reference Base for Soil Resources
- ↑ http://www.bbc.co.uk/sn/tvradio/programmes/horizon/dino_prog_summary.shtml
- ↑ http://science.jrank.org/pages/2643/Extinction-asteroid-impact-theory.html
- ↑ The Sedimentary Record of Meteorite Impacts - Kevin R. Evans
- ↑ http://www.pwrc.usgs.gov/set/installation/markers.html
- ↑ Pool, p. 181.
- ↑ van der Plicht J; Hogg A (Dec 2006). "A note on reporting radiocarbon". Quaternary Geochronology 1 (4): 237–240. doi:10.1016/j.quageo.2006.07.001. http://www.sciencedirect.com/science?_ob=ArticleURL&_udi=B83WJ-4KXF320-1&_user=10&_coverDate=12%2F31%2F2006&_rdoc=1&_fmt=high&_orig=search&_origin=search&_sort=d&_docanchor=&view=c&_acct=C000050221&_version=1&_urlVersion=0&_userid=10&md5=1801c6a21ef32b482a1447c290659d82&searchtype=a.
- ↑ A. Mulch; A. M. Sarna-Wojcicki; M. E. Perkins; C. P. Chamberlain (13 May 2008). "A Miocene to Pleistocene climate and elevation record of the Sierra Nevada (California)". Proceedings of the National Academy of Sciences of the United States of America 105 (19): 6819–6824. doi:10.1073/pnas.0708811105. http://www.pnas.org/content/105/19/6819.full. Retrieved 2017-06-11.
- ↑ Mount St. Helens National Volcanic Monument. USDA Forest Service. Retrieved 2006-11-26.
- ↑ May 18, 1980 Eruption of Mount St. Helens. USDA Forest Service. Retrieved 2007-08-11.
- ↑ Mullineaux, The Eruptive History of Mount St. Helens, USGS Professional Paper 1250, page 3
- ↑ USGS Description of Mount St. Helens, USGS.gov . Retrieved 15 November 2006.
- 1 2 3 Bonadonna C; Ernst GGJ; Sparks RSJ (May 1998). "Thickness variations and volume estimates of tephra fall deposits: the importance of particle Reynolds number". J Volcanology Geothermal Res 81 (3–4): 173–187. doi:10.1016/S0377-0273(98)00007-9. http://www.geo.mtu.edu/~raman/papers2/Bonadonnaetal1998JVGR.pdf.
- 1 2 Schuster PF; Krabbenhoft DP; Naftz DL; Cecil LD; Olson ML; DeWild JF; Susong DD; Green JR et al. (Jun 2002). "Atmospheric mercury deposition during the last 270 years: a glacial ice core record of natural and anthropogenic sources". Environ Sci Technol. 36 (11): 2303–2310. doi:10.1021/es0157503. PMID 12075781. http://webhost.ua.ac.be/mitac4/instr/Hg_270years_EnvSciTech_36_2002_2303_2310.pdf.
- 1 2 3 4 5 6 7 8 9 10 11 Mullineaux DR (1996). "Pre-1980 Tephra-Fall Deposits Erupted From Mount St. Helens, Washington". USGS Professional Paper (1563). http://vulcan.wr.usgs.gov/Volcanoes/MSH/EruptiveHistory/summary_msh_eruptive_stages.html.
- 1 2 Hallett DJ; Mathewes RW; Foit FF Jr (May 2001). "Mid-Holocene Glacier Peak and Mount St. Helens We Tephra Layers Detected in Lake Sediments from Southern British Columbia Using High-Resolution Techniques". Quart Res. 55 (3): 284–292. doi:10.1006/qres.2001.2229. http://www.nau.edu/~envsci/DJHallett/downloads/QR01Hallett.pdf.
- ↑ Fiacco RJ Jr; Palais JM; Germani MS; Zielinski GA; Mayewski PA (1993). "Characteristics and possible source of a 1479 A.D. volcanic ash layer in a Greenland ice core". Quart Res 39 (3): 267–273. doi:10.1006/qres.1993.1033.
- 1 2 LaMarche VC Jr; Hirschboeck KK (Jan 1984). "Frost rings in trees as records of major volcanic eruptions". Nature 307 (5946): 121–126. doi:10.1038/307121a0. http://fp.arizona.edu/kkh/nats101gc/PDFs-09/LaMarche.Hirschboeck.1984.all.edt.opti.pdf.
- ↑ LaMarche VC Jr; Harlan TP (1973). "Accuracy of Tree Ring Dating of Bristlecone Pine for Calibration of the Radiocarbon Time Scale". J Geophys Res. 78 (36): 8849–8858. doi:10.1029/JC078i036p08849.
- ↑ Simkin T; Siebert L; McClelland L; Bridge D; Newhall C; Latter JH (1981). Volcanoes of the World. Stroschberg: Hutchinson Ross.
- ↑ Degens, E.T.; Buch, B (1989). "Sedimentological events in Saleh Bay, off Mount Tambora". Netherlands Journal of Sea Research 24 (4): 399–404. doi:10.1016/0077-7579(89)90117-8.
- ↑ Oppenheimer, Clive (2003). "Climatic, environmental and human consequences of the largest known historic eruption: Tambora volcano (Indonesia) 1815". Progress in Physical Geography 27 (2): 230–259. doi:10.1191/0309133303pp379ra.
- ↑ Dansgaard W. Frozen Annals Greenland Ice Sheet Research. Odder, Denmark: Narayana Press. p. 124. ISBN 87-990078-0-0.
Further reading
- Dansgaard W. Frozen Annals Greenland Ice Sheet Research. Odder, Denmark: Narayana Press. p. 124. ISBN 87-990078-0-0.